Welcome to Complex Liquid Thermoelectrics Research group
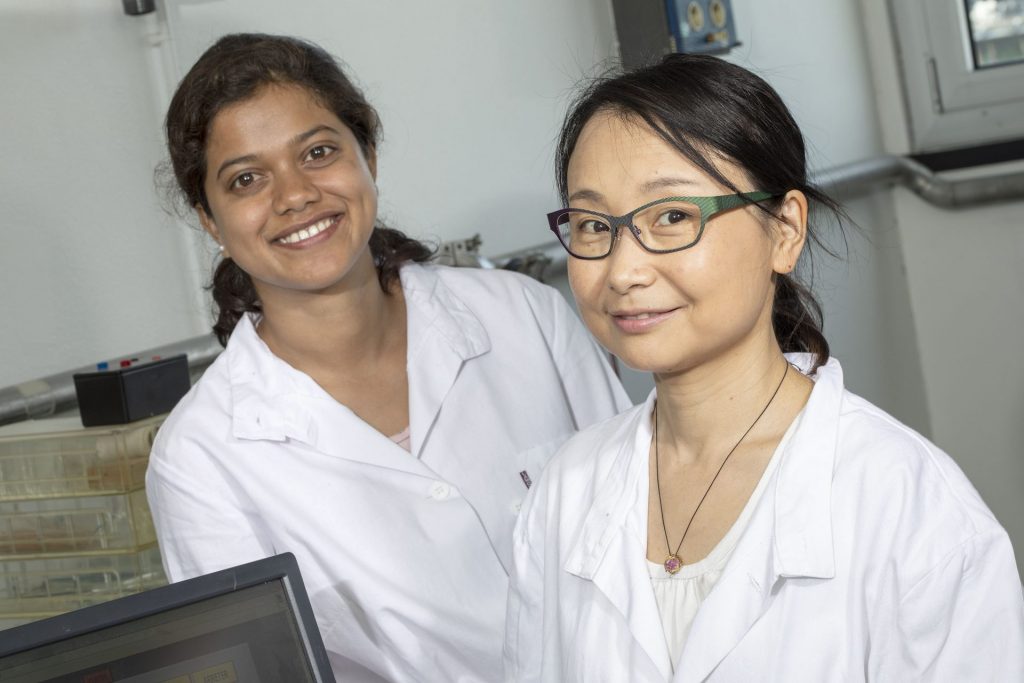
NEW!!! Internship position (4-6 months, experimental) for thermally chargeable supercapacitor develoment and characterization
M2 level, starting Feb/March 2020. Possible continuation as a PhD candidate from Fall 2020.
To know more click HERE
Who & What
We study thermal-to-electrical energy conversion phenomena in liquids by combining experimental techniques from physics, physical chemistry and electrochemistr. It is an exciting new field of research that is attracting increased attention as an alternative solution to semi-conductor based thermoelectric materials. The underlying physics , the types of complex liquids and possible future application paths are described below.
Also….
Please visit our MAGENTA project page https://www.magenta-h2020.eu
A member of NANOUPTAKE– Overcoming Barriers to Nanofluids Market Uptake (COST Action CA15119)
Thermoelectricity at a glance
The application of a temperature difference ΔT across a solid conductor causes mobile charge carriers to diffuse from hot to cold region, giving rise to a thermoelectric voltage V = -Se∆T. The prefactor Se is called th thermopower or Seebeck coefficient since the discovery of this phenomenon by Seebeck in 1821. This enables the conversion of heat to electricity.
In ordinary metals like copper, the Seebeck coefficient Se is of the order of 10 μV/K. In the mid 20th century much higher Seebeck coefficients of a few hundreds of μV/K were obtained in low-gap semi-conductors, and those materials are still the object of intense research activities, with the perspective of converting low-grade wasted heat; e.g., body heat, car exhausts, industrial waste streams, solar heating, etc., into electric energy. Conversely, the application of voltage ΔV results in a temperature difference (the Peltier effect) such that the same material can be used as a thermoelectric (TE)-cooler.
Despite the robustness of TE-technology (including long life-time, no moving parts, etc.) and its wide range of applicability (wherever a thermal gradient exists), the TE-generators market has so far been restricted to a small number of technological areas due to their low efficiency. The thermal-to-electrical energy conversion efficiency, η of a given TE device is most often expressed as a function of a dimensionless parameter ZT, called “figure of merit”:
ZT = TSe2(σ/κ) (1).
ZT is proportional to the square of the Seebeck coefficient Se and to the ratio between the electrical (σ) and thermal (κ) conductivities. Up until 20 years ago, TE-devices had been based almost exclusively on low-gap solid-state bulk semiconductors whose ZT values are comprised in the ~0.1 – 1.0 range [1]. Compared to other heat engines (see Figure 1), there is no wonder why the TE-technology was not considered for a wider and larger-scale heat recovery applications.
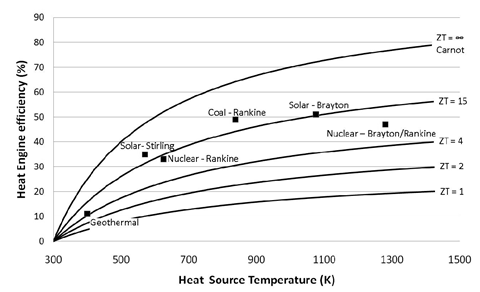
Figure 1: TE-conversion efficiency as a function of ZT and heat source temperature compared to other heat engine technologies. Image taken from [2]
In the mid-1990’s Hicks et al., [2] predicted significant improvements in the thermoelectric efficiency in nanoscale elements. This has generated a great surge in nanostructured thermoelectric (micro)generators (See figure (left)). In a very simplified term, by ‘nano’structuring, one can reduce the material’s thermal conductivity κ, without affecting its electrical conductivity σ, resulting in an enhancement of ZT, figure of merit (see Eq. (1)).
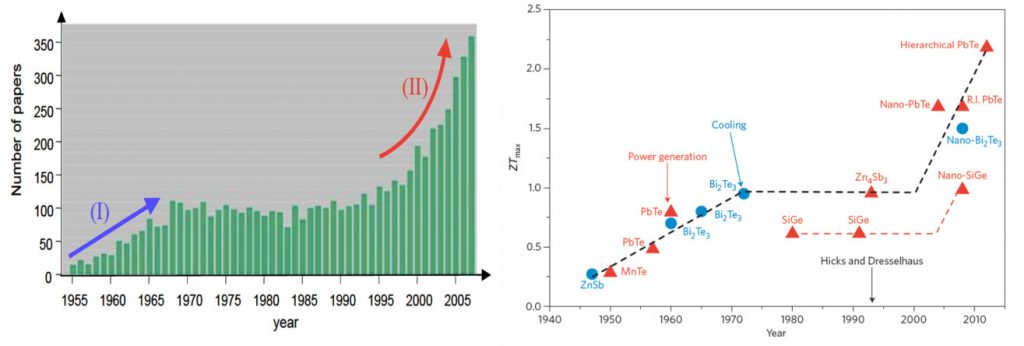
Figure 2: (Left) Publication trend in thermoelectric research [3] and (Right) Historical progress in ZT values [4].
High ZT values (over 2 at room temperature, see Figure 2 right panel) made of bismuth-telluride and its alloys have been reported. Unfortunately, these nanostructured devices are limited to very small active surface areas and incur huge production costs. Furthermore nearly all high-performance TE materials contain rare and often toxic raw materials (high scarcity and health risks) [5], highlighting a need for a transformation thermoelectric technology made with less critical material.
Thermoelectric effects in liquid electrolytes
Liquid electrolytes are characterised by the presence of several ion species (dissolved in a liquid matrix) and their « thermoelectric » effects have been known since the end of the 19th century. The observed TE-coefficients in electrolytes are generally an order of magnitude larger that the semiconductor counterparts, including nanostructured materials (see Figure 3 below). The electrical conductivity of these liquids, however, is a few orders of magnitude lower than solid counterparts and therefore, liquid based TE-systems have long been considered technologically irrelevant despite their advantages; e.g., material abundance, low production costs, etc..
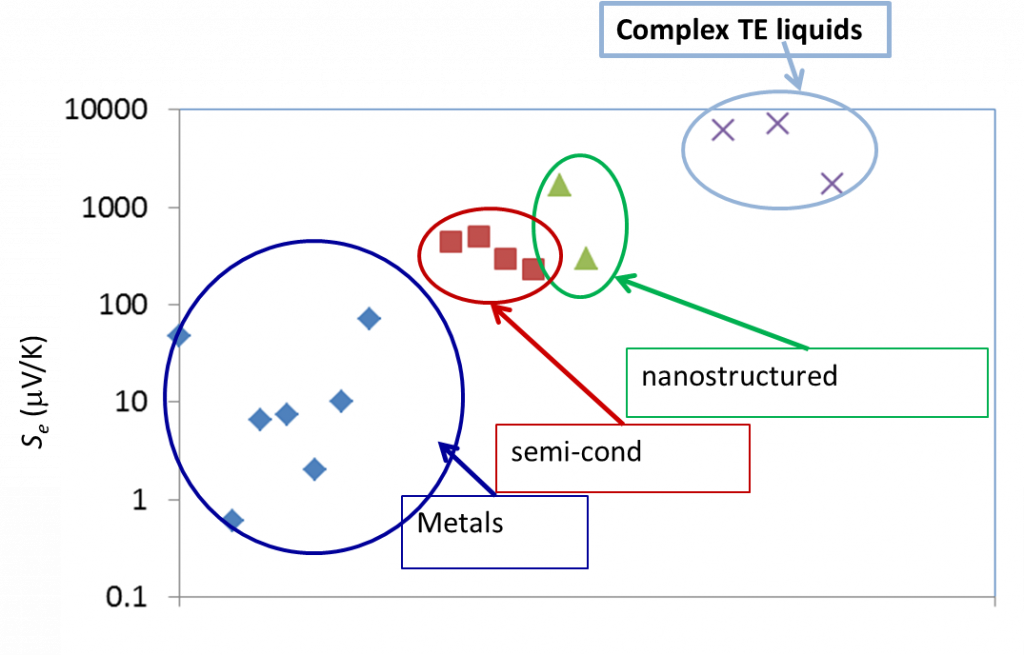
Figure 3: Comparison of TE voltage (open circuit) of different materials
Since the discovery of TE-effects in complex liquids such as ionic-liquids/solvent binary mixtures, and charged colloidal and macromolecular suspensions, such perception surrounding liquid thermoelectrics is changing in recent years. Broadly speaking, there are 3 different sources of thermoelectric power in electrolyte containing thermoelectric cells; namely, (a) Thermogalvanic effect b) thermodiffusion of ionic species and c) Selective ion attachments of ionic species to the hot and cold electrodes.
- Thermogalvanic effect: Also known as 'thermoelectrochemical effect,’ relies on redox molecules to reversibly exchange electrons (oxidation-reduction mechanism) with hot-and cold electrodes to produce large reaction entropy change; i.e., thermogalvanic-voltage. The redox couples not only serve to produce ‘voltage’ but are responsible for producing electric current in a thermocells by exchanging electrons with hot and cold electrodes. Typically observed Seebeck coefficient in thermogalvanic cells containing ILs and other liquid electrolytes is about 1 mV/K an order of magnitude larger that the semiconductor counterparts, including nanostructured materials.
- Thermodiffusion effect: The TE-effect in complex liquids (electrolytes, ionic liquids, colloidal solutions etc.) is further coupled to the movement of dissolved charged species (ions, molecules or colloidal particles) and is closely related to the “Soret effect” which describes the concentration gradients, ni induced by a temperature gradient; (Δni/ni) = αiΔT, where a is called “Soret coefficient.” The Soret effect or “thermophoresis” has been extensively studied in the last decades [16-18] and is often used for microfluidic characterization and separation. The Soret coefficient, a, is generally proportional to the entropy transported by the moving molecules or particles (i.e., internal degrees of freedom, large solvation shell, particle/molecule size, etc.). Therefore, large thermoelectric coefficients can be expected in complex liquids exhibiting large values of .
- Electronic double layer (EDL): Closely related to the thermodiffusion effect described above, the assymetric distribution of ionic species at the hot and cold electrodes can result in the temperature dependent electronic double layer capacitance at the liquid/electrode interfaces.
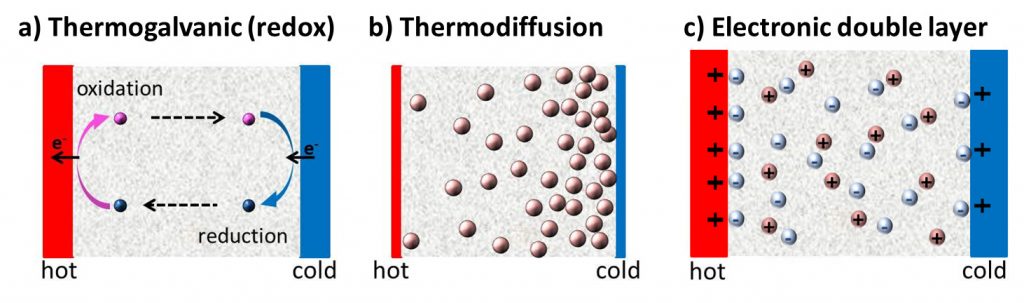
Figure 4: Different mechanisms at the origin of thermoelectric voltage in liquid electrolytes.
While these effects are independently known to exist in liquid electrolytes and complex liquids (such as colloidal suspensions and ionic liquids), the interdependency among thermogalvanic-thermodiffusion-EDL effect is still in the basic research phase. At SPHYNX, we are exploring these novel thermoelectric phenomena in various complex liquids.
We focus on ….
- Non-aqueous electrolytes: We have measured thermoelectric power in various non-aqueous electrolytes (alocohols, organic solvents) where TE voltage as high as 7mV/K has been measured [7]
- Ionic liquids: We investigate all 3 TE effects either in TE-generator or in TE-capacitor mode. [8, 9]
- Ferrofluids: In 2015, we have reported a first-of-its-kind experimental study on the coupled thermodiffusion and thermoelectric effects in ferrofluids based on organic solvents [10] and on water [11]. This work served as a true proof-of-principle, demonstrating the contribution of thermodiffusion of charged colloidal particles to the total thermoelectric voltage of the carrier fluid. Experimental parameters such as magnetic volume fraction, particle size, applied magnetic field strength and its direction that can be tuned to control the TE property of ferrofluids. The magnetic nature of particles further encourages the use of ferrofluids for magneto-thermoelectric energy conversion applications.
- Develop novel thermoelectric measurement techniques at high (above 150°C) and low (below 0°C) temperatures and under magnetic field.
![]() |
![]() |
![]() |
![]() |
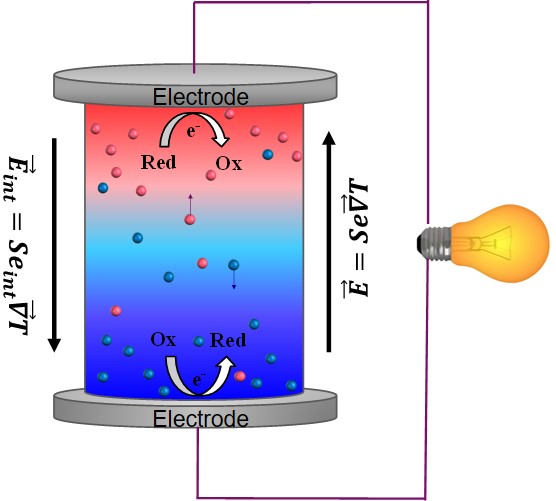
References:
[1] F. J. DiSalvo, « Thermoelectric Cooling and Power Generation, » Science, vol. 285, pp. 703-706, 1999.
[2] C. B. Vinning, « An Inconvenient Truth About Thermoelectrics, » Nature Materials, vol. 8, p. 83, 2009.
[3] L. D. Hicks and M. S. Dresselhaus, « Effect of quantum-well structures on the thermoelectric figure of merit, » Phys. Rev. B, vol. 47, p. 12727–12731, 1993.
[4] M. W. Gaultois et al., « Date-driven review of thermoelectric materials: Performance and resource considerations, » Chmistry of Materials, vol. 25, p. 2911, 2013.
[5] J.-C. Zheng, « Recent advances on thermoelectric mateirals, » Frontiers of Physics in China, vol. 3, no. 3, p. 269, 2008.
[6] J. P. Heremans et al., « When thermoelectrics reached the nanoscale, » Nature Nanotechnology, vol. 8, p. 471, 2013.
[7] M. Bonetti et al., « Huge Seebeck coefficient in non-aqueous electrolyte, » J. Chem. Phys., vol. 134, p. 114513, 2011.
[8] V. Zinovyeva et al., « Enhanced thermoelectric power in ionic liquids, » ChemElectroChem, vol. 1, p. 426, 2014.
[9] M. Bonetti et al., « Thermoelectric energy recovery at ionic-liquid/electrode interface, » The Journal of Physical Chemistry, vol. 142, p. 244708, 2015.
[10] B. T. Huang et al., « Thermoelectricity and thermodiffusion in charged colloids, » The Journal of Chemical Physics, vol. 143, p. 054902, 2015.
[11] T. Salez et al., « Can charged colloidal particles increase the thermoelectric energy conversion efficiency? » Phys. Chem. Chem. Phys. 19 (2017), 9409.
Funding sources: FET Proactive H2020 2017 MAGENTA : « MAGnetic nanoparticle based liquid ENergy materials for Thermoelectric device Applications » (see CORDIS) – Grant No. 731976, 2017-2021, (coordinator) CNRS-Projets exploratoires (Energie) NOTURNE: « Nouvelle génération de cellules thermogalvaniques : conception, développement et performance » 2016-2017 (partner) ANR-PROGELEC « TE-FLIC » Thermoélectricité dans des ferrofluides, liquides ioniques et colloïdes (Grant No. ANR-12-PRGE-0011-01) CEA program « Flûte » Fluides thermoélectriques (CEA/DSM-ENERGIE), LabEx PALM (No. ANR-10-LABX-0039-PALM): « Magneto-thermoelectric power in ferrofluids » (MAGTEP) : New thermoelectric energy conversion material » To know more, please contact: Sawako Nakamae