The characterization of paramagnetic species within a sample using Electron Spin Resonance Spectroscopy (ESR) has many applications in chemistry, biology, archaeology and dosimetry. This 80-year-old technique consists of measuring the absorption of microwave radiation by electron spins at their resonance frequency, using a resonator for detection.
For the past 10 years, SPEC’s quantronics team and its collaborators have been developing a research program that aims to use the extreme sensitivity of superconducting circuits in the quantum regime (cooled to 10 mK) to perform ESR spectroscopy of a single spin. Following successive improvements in sensitivity, this ultimate goal has now been achieved, using a microwave photon counter based on a superconducting transmon qubit.
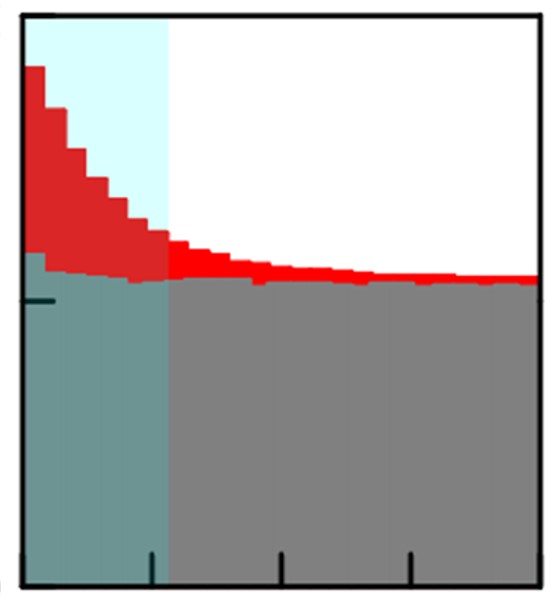
The weak point of ESR spectroscopy is its limited sensitivity, which typically requires at least a milliard of spins to detect a signal. This prevents the method from being applied to small samples, such as micro-crystals, individual cells, and a fortiori individual molecules. In addition, the resonance frequency of spins is generally very sensitive to their electrostatic and magnetic environment, and thus differs slightly from one spin to another. The spectral width of an ESR resonance line is thus dominated by this inhomogeneous broadening and can reach several GHz, thus limiting the achievable spectral resolution.
The ultimate way to overcome this inhomogeneous broadening is to measure the response of individual spins, but this requires the development of an ESR method that is sensitive to a single spin, compatible with all electron spins, within a detection volume that is not too small [1].
The principle of the experiment is as follows (see figure): a superconducting LC microwave circuit is fabricated on a crystal containing the spins to be detected. The microwave current passing through the wire, which acts as an inductor, generates a magnetic field that couples to the nearest spins. Frequency tuning to the resonance of an identified spin is achieved by applying a variable magnetic field B0 in the plane of the sample. A microwave pulse sent to the resonator excites this spin, which then relaxes back to its ground state with relaxation rate ΓR, re-emitting a microwave photon, which is then detected with efficiency 0 < η < 1 by the microwave photon counter [2]. The latter is not perfect, however, and has a false positive rate α, in the form of “dark hits”.
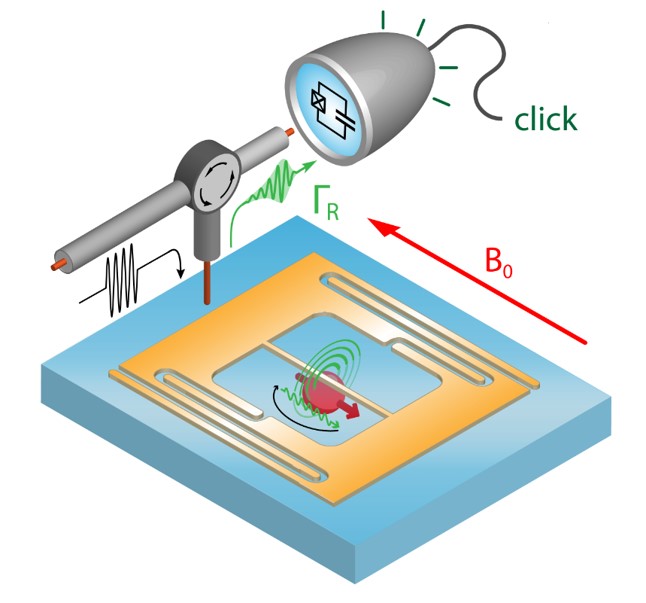
Principle of single spin detection. Er3+ ions carrying a spin (red) are implanted in a CaWO4 crystal (blue). By applying a magnetic field B0 the spin is tuned to resonance at 7.334 GHz with the mode of an LC resonator (orange) directly fabricated on the crystal. The spin excited by a pulse relaxes with a rate ΓR by emitting a microwave photon, detected by a microwave photon counter based on a transmon-type qubit.
In a first experiment in 2021 [3], the method was shown to detect a signal from ∼104 spins. To achieve the sensitivity needed to detect a single spin, a signal-to-noise ratio must be reached, such that the number of hits from the spin to be detected is at least of the same order as the number of dark hits. One way of achieving this ultimate sensitivity is to increase ΓR as much as possible by optimizing the resonator geometry to enhance the Purcell effect (see note*) [3,4]. The system chosen consists of Er3+ erbium ions, selected for their high magnetic moment, and implanted in a CaWO4 crystal. Finally, a new generation of ultra-low-noise photon counter has been developed, making it possible to reach α ∼ 100 s-1, and thus the single-spin regime η ΓR ≈ α.
To measure the spectrum of this sample, the average number of counts detected during the 2 milliseconds following an excitation pulse is recorded as a function of the magnetic field B0 (see Fig.1b). A broad peak centered at 419.5 mT is observed, corresponding to the magnetic resonance of an ensemble of Er3+:CaWO4 ions [5], but also a series of very narrow peaks, each corresponding to the emission of an individual ion.
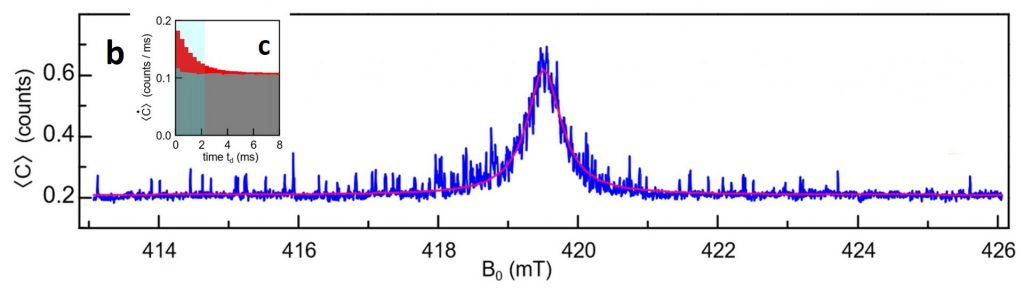
(b) ESR spectroscopy: number of average counts detected <C> by the counter, following the excitation pulse as a function of the B0 field (blue curve): the broad peak at 419.5 mT is the ESR resonance of all Er3+ ions (the red profile is a fit by a Gaussian). The narrow peaks come from individual Er3+ ions in different environments. Inset shows the emission for one of these spins, which has a relaxation rate ΓR ∼ 650 s-1.
Observing the Rabi oscillations of the excited spins and measuring their amplitude gives a measure of the detection efficiency η = 0.12. Proof that an individual spin is indeed detected is obtained by measuring the correlation function g(2)(k): as expected g(2)(k) ∼ 1 when k ≠ 0, and g(2)(0) = 0.2 ± 0.1. This indicates that it is highly unlikely to obtain 2 photons simultaneously during the same microwave excitation sequence, and that the photons detected do indeed originate from a single Er3+:CaWO4 spin.
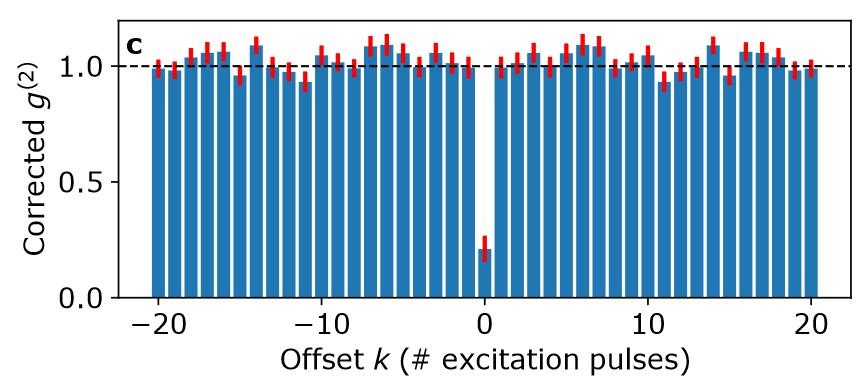
Autocorrelation function g(2)(k) corrected for dark noise, where k is the number of excitation sequences between two measurements. The measurement g(2)(k) = 0.2 ± 0.1 proves that the emission measured during a single sequence does indeed come from a single spin.
This result shows that it is now possible to detect the magnetic resonance signal of one single electron spin using quantum superconducting circuits. The method developed is directly applicable to a large number of systems, offers a large detection volume (10 μm3), and thus brings us closer to the goal of performing single-spin magnetic resonance spectroscopy operationally. Research is continuing into applications of the method to quantum computing with multiple robust spin qubits, as well as to ESR spectroscopy with a view to measuring individual molecules.
* The Purcell effect is a coupling effect between an emitter (two-level system) and the modes of the cavity to which it is coupled. When the frequency of the emitter is in resonance with the cavity, its spontaneous emission is exalted, and the radiative emission rate ΓR is therefore considerably increased, compared with the same emitter placed in free space outside the cavity (in the experiment carried out, the “Purcell factor” reached 1015!).
References:
[1] “Single electron-spin-resonance detection by microwave photon counting”,
Z. Wang, L. Balembois, M. Rančić, E. Billaud, M. Le Dantec, A. Ferrier, P. Goldner, S. Bertaina, T. Chanelière, D. Estève, D. Vion, P. Bertet, E. Flurin, arxiv:2301.02653 (2023) – Nature 619, (2023) 276–281.
[2] “Irreversible Qubit-Photon Coupling for the Detection of Itinerant Microwave Photons”,
R. Lescanne, S. Deléglise, E. Albertinale, U. Réglade, T. Capelle, E. Ivanov, T. Jacqmin, Z. Leghtas, and E. Flurin, Physical Review X 10, 021038 (2020)
[3] “Detecting spins by their fluorescence with a microwave photon counter”,
E. Albertinale, L. Balembois, E. Billaud, V. Ranjan, D. Flanigan, T. Schenkel, D. Estève, D. Vion, P. Bertet, E. Flurin, Nature 600, 434 (2021)
[4] “Controlling spin relaxation with a cavity”,
A. Bienfait, J. J. Pla, Y. Kubo, X. Zhou, M. Stern, C. C. Lo, C. D. Weis, T. Schenkel, D. Vion, D. Esteve, J. J. L. Morton, P. Bertet, Nature 531, 74 (2016)
[5] “Twenty-three–millisecond electron spin coherence of erbium ions in a natural-abundance crystal”,
M. Le Dantec, M. Rančić, S. Lin, E. Billaud, V. Ranjan, D. Flanigan, S. Bertaina, T. Chanelière, P. Goldner, A. Erb, R. B. Liu, D. Estève, D. Vion, E. Flurin, P. Bertet,, Science Advances, 7 (2021) eabj9786, arXiv:2106.14974.
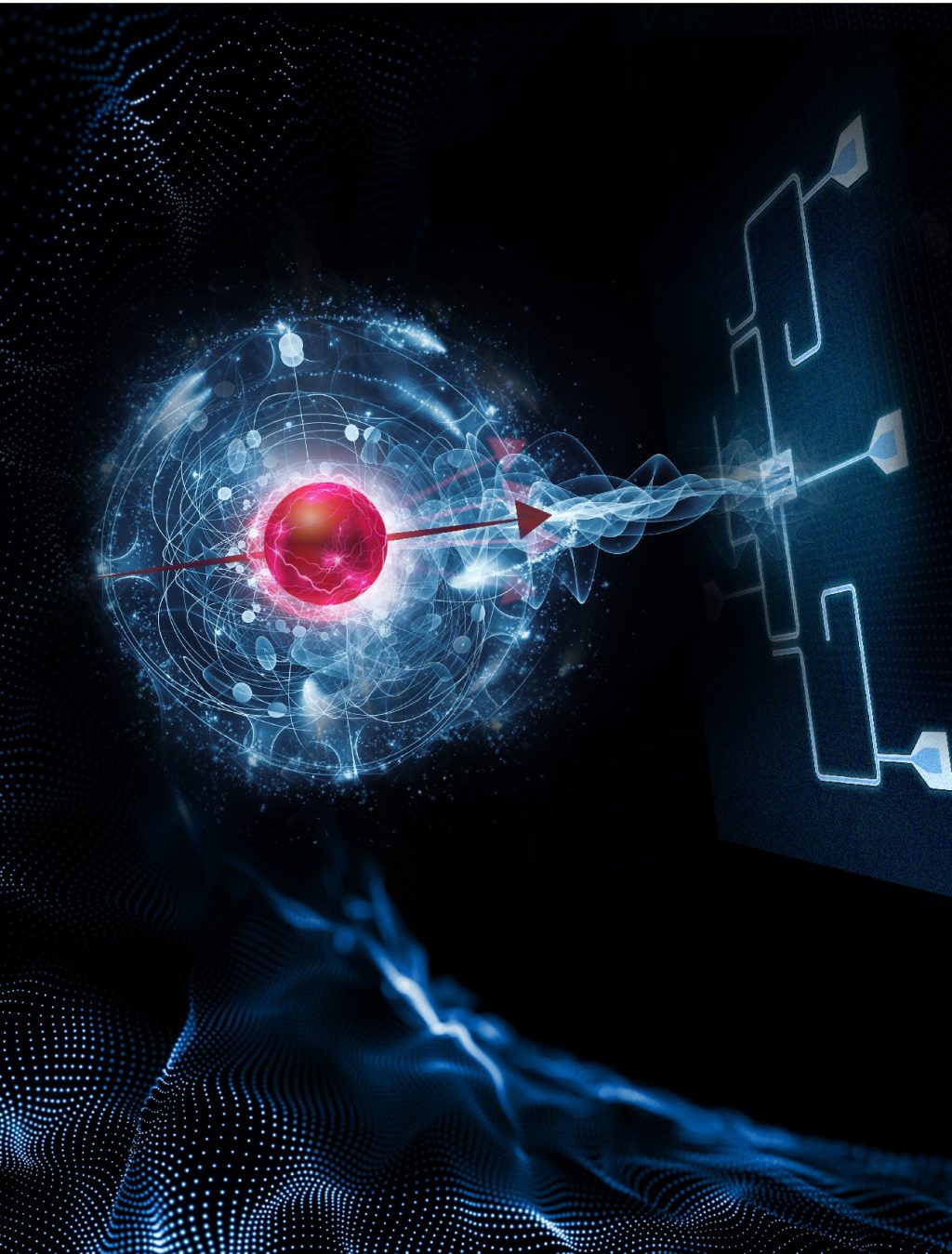
Previous highlights:
- RPE : Détection de de la réponse de spins individuels avec un capteur de photon unique (Décembre 2021)
- Contrôler la relaxation de spin avec une cavité (Février 2016)
Contact CEA: Patrice Bertet (SPEC/Quantronique)
Collaboration:
- Quantronics group, Université Paris-Saclay, CEA, CNRS, SPEC, 91191 Gif-sur-Yvette Cedex, France
- Département de Physique et Institut Quantique, Université de Sherbrooke, Sherbrooke, Québec, Canada
- Chimie ParisTech, PSL University, CNRS, Institut de Recherche de Chimie Paris, 75005 Paris, France
- CNRS, Aix-Marseille Université, IM2NP (UMR 7334), Institut Matériaux Microélectronique et Nanosciences de Provence, Marseille, France.
- Univ. Grenoble Alpes, CNRS, Grenoble INP, Institut Néel, 38000 Grenoble, France