Ultrafast materials science
Our group is interested in ultrafast materials science, and more specifically in the study of solids using the shortest light sources available today: attosecond pulses (1as = 10-18 s). These pulses are obtained by generating high-order harmonics in gases. Here’s an example presented in both time and frequency domains. Here, the pulse is generated in a gas cell filled with argon :
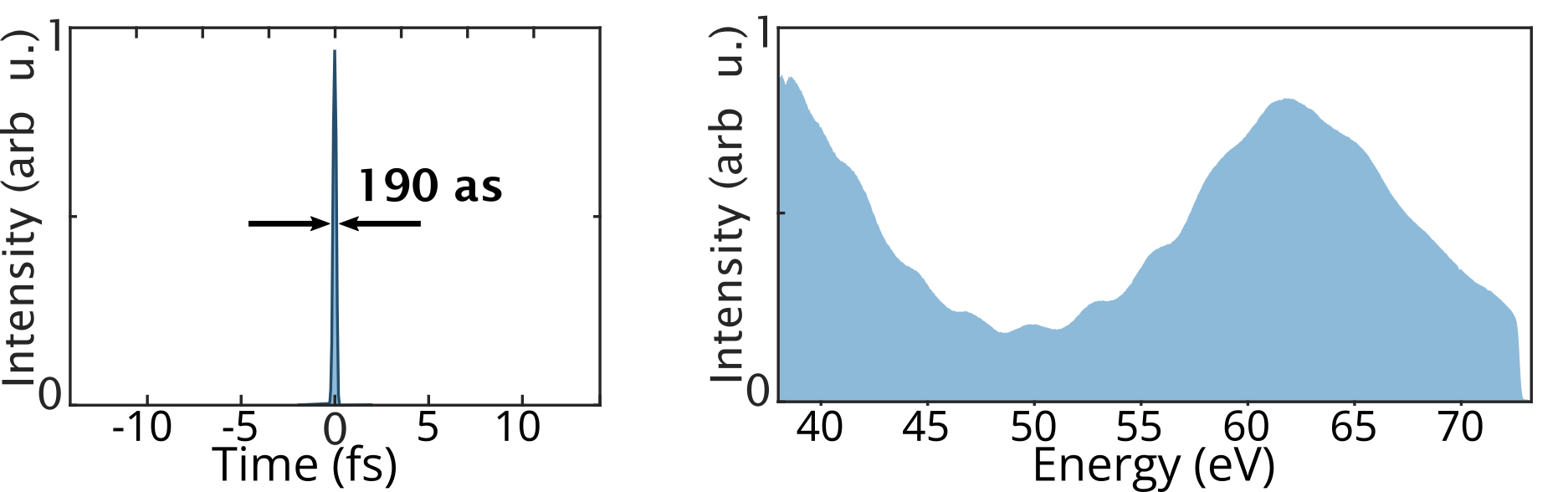
Attosecond pulses have two essential properties:
1 – Exceptionally short durations. This makes it possible to capture electronic dynamics, which generally occur in a few femtoseconds. Notably, experimental resolution can now be much lower than an optical cycle of laser light.
2 – A broad spectral band with wavelengths in the extreme ultraviolet (XUV) and even X-rays. The energies of these photons resonate with transitions from the core levels of sample atoms to their valence and conduction bands. As a result, the dynamics can be probed from each atom’s “point of view”. In a sense, this enables attosecond spectroscopy to see what’s going on “inside” a material.
Transient absorption and reflectivity on the attosecond scale
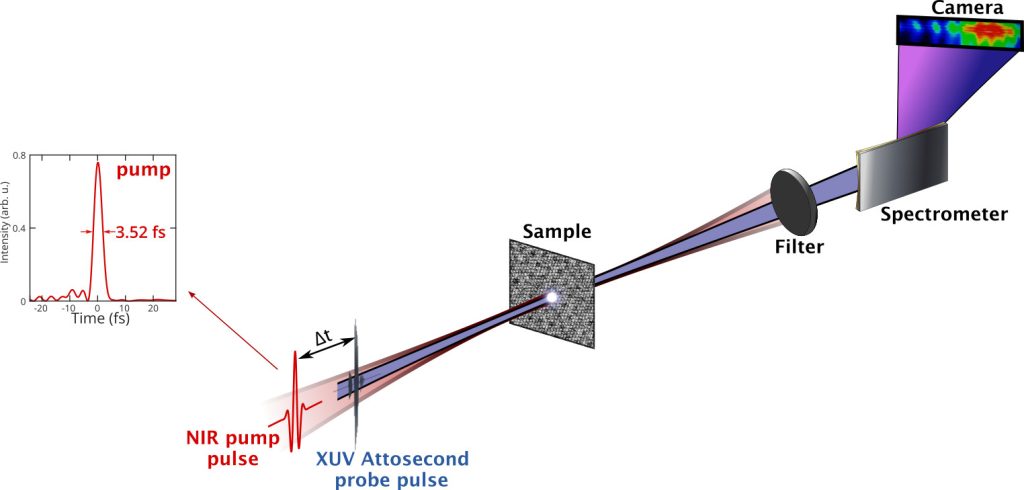
We carry out experiments on LIDYL’s ATTOLab platform. We operate a light line dedicated to solid-state spectroscopy.
In these experiments, the sample is first excited by a short optical pulse (
Remarkably, this technique is entirely optical, meaning that its temporal (set by pulse duration) and spectral (set by spectrometer resolution) resolutions can be optimally short and narrow respectively.
Topics of interest
High-field physics in dielectrics
Dielectrics are transparent materials: under weak excitation, they absorb no energy from the pump beam.
However, when the laser’s electric field becomes sufficiently strong, it is possible to trigger numerous effects involving changes in band structure (see drawing below) and electron dynamics (e.g. tunneling, avalanche ionization).
These strong-field phenomena can be spectacular; for example, short pulses of light can increase the AC conductivity of a solid by 18 orders of magnitude in less than a femtosecond, and set electrons and holes in oscillation at frequencies in the Petahertz range.
Attosecond spectroscopy allows us to observe how these processes unfold, revealing how powerful laser pulses can manipulate materials on sub-femtosecond timescales, possibly reversibly.
Read more
Understanding these high-intensity phenomena is also important because the interaction between light and dielectrics is ubiquitous in various applications in optics and electronics.
For example, field-induced currents in dielectrics are the fundamental phenomenon behind the generation of high harmonics in the solid state, which is also studied in the DICO group.
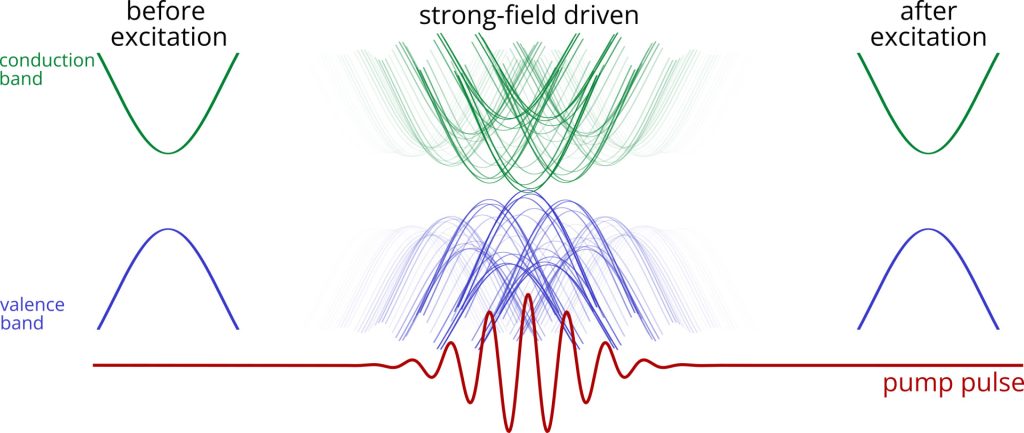
Coherent light-spin interactions
The control of electrons (i.e. electron charge) by light is increasingly well understood at the attosecond scale.
However, the control of electron spin is much less developed. Most electron spin control systems rely on indirect processes: light first excites the electrons, which then relax as a function of spin (visual “a” below).
This is the basis of femtosecond laser-induced demagnetization, but only leads to relatively slow spin responses.
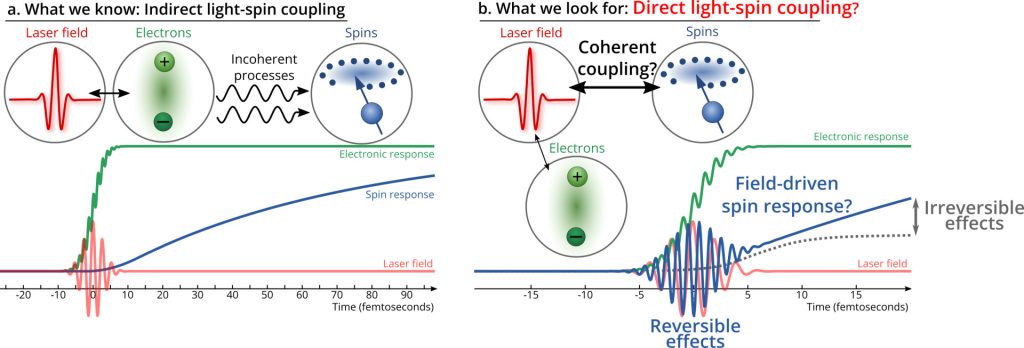
Interestingly, several reports have provided evidence that more direct and coherent light-spin interactions may exist (visual “b” above).
Verifying these theories is very difficult: we need to adapt attosecond spectroscopy to probe spin dynamics in materials at sub-femtosecond time scales, which has never been done before.
One of our projects aims to do just that. We aim to carry out XUV magnetic circular dichroism experiments with attosecond pulses, while maintaining a time resolution of less than 100.
Ultrafast dynamics in highly correlated materials
We have recently begun studying a new class of solids: highly correlated materials.
This broad category of samples generally exhibits unusual (and often useful) electronic and magnetic properties. Their common feature is that these properties cannot be properly described in terms of interacting electrons or spins.
Instead, electronic correlations are an essential and defining feature of these solids.
The result is a wide range of effects: light-induced phase transitions, (anti)-ferromagnetism, multiferroism and even superconductivity.
Although we are a long way from studying phenomena such as superconductivity with attosecond spectroscopy, we can already investigate some fascinating questions. As an example, consider the sketch in panel (a) of the following figure:
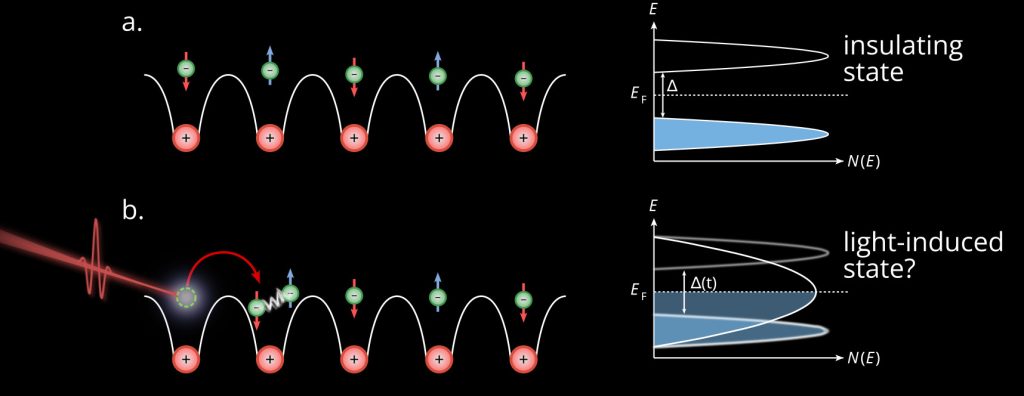
Such a state is obtained when the interaction between electrons is strong: there is one electron per atom, with antiferromagnetic order, which cannot move due to the strong repulsion. This is known as a Mott insulator.
When such a system is excited with a short laser pulse (see panel (b)), this fragile state is broken, as the added energy overcomes the electron repulsion.
This leads to a complex time-dependent state; for example, the material can instantly become metallic!
This light-induced response is governed purely by electronic correlations, and is therefore extremely rapid.
We have begun to use attosecond spectroscopy to probe the behavior of correlated laser-excited insulators on previously unexplored time scales. Our aim is to understand how correlation influences the strong-field response.
In general, the time-domain response could be a valuable probe of the importance of electronic correlation in any material.
Attosecond photoemission delays in solids
While all the above topics use absorption or reflection measurements (“photon-in, photon-out”), we also perform photoemission spectroscopy in the solid state (“photon-in, electron-out”).
We operate a second beamline, equipped with an angle-, spin- and time-resolved photoelectron spectrometer (ARPES), in collaboration with our partners at LPMS.
The difficulty with photoemission lies in the fact that we cannot easily use broadband attosecond pulses: these would lead to electron wave packets with a bandwidth of several eVs, which would destroy the energy resolution of the experiment.
This is why most ARPES experiments focus on the >30 fs range.
Another approach is to adapt gas-phase attosecond photoemission techniques. In particular, our colleagues in the ATTO group are experts in the RABBIT technique, which uses interference between several narrow harmonics to measure the time taken for an electron to emerge from an atomic or molecular potential.
These so-called photoemission delays are typically of the order of tens of attoseconds.
We are currently conducting new experiments aimed at adapting this technique to solids. This is a technically challenging task, since it requires time stabilities to be maintained for many hours.
If successful, solid-state photoemission delays could become a new type of observable providing information on electronic properties.