Introduction
For over 15 years, our group has been interested in plasma mirrors, an object of study for the fine coupling between lasers with short, intense pulses and matter. At high intensities (>1016W/cm2), they generate attosecond UV-X radiation sources, in the specular direction of the incident laser, the study of which provides information on the ultrafast dynamics of the plasma created. They can also,
as such, to generate particle sources, in particular high-charge relativistic electron packets, and coupled with a gas source to produce application-quality injectors. The coupling of experiments and simulations has led to major results in the field, the most recent of which are presented below.
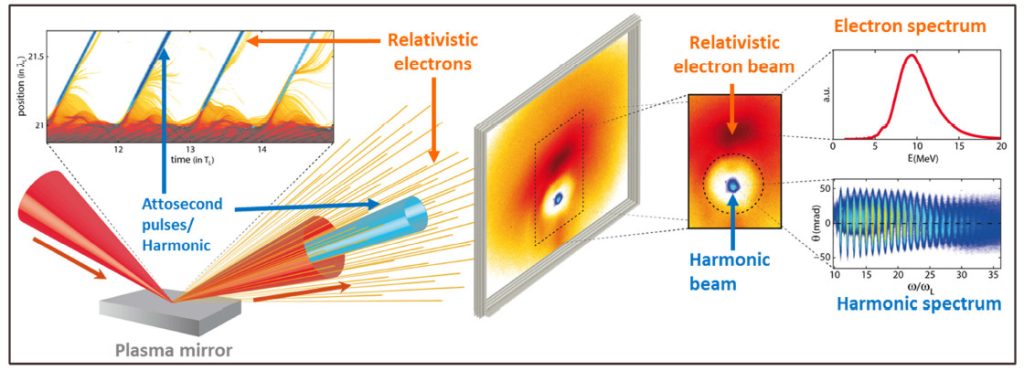
schematic representation of plasma mirror physics
When interacting with an intense laser beam, plasma mirrors reflect the incident laser beam in the specular direction with a reflectivity of over 70% (bottom left). The highly non-linear coupling with the field (top left image-extracted from PIC simulations) leads to the emission of high-order harmonic beams and relativistic electrons. A typical image of these beams is shown in the center, and their respective spectral distribution in the images on the right (taken from experiments on the UHI100 platform)
Plasma mirrors are also being considered as devices for boosting the laser intensity of current PW systems, in the hope of one day reaching the Schwinger limit and studying strong-field quantum electrodynamic processes.
Chaotic dynamics
A collaboration between the LIDYL team at CEA Saclay and the ATP team at Lawrence Berkeley National Lab (LBNL) has elucidated the absorption mechanisms of an ultra-intense laser beam as it reflects off a dense plasma formed on the surface of a solid target.
These mechanisms, previously unidentified for laser intensities >1018 W.cm-2, are involved in numerous laser-plasma processes, such as the production of relativistic electron and ion beams
and ions, or short-wavelength light beams (emission of high-order Doppler harmonics), with multiple applications (spectroscopy, irradiation, medicine, etc.).
A detailed understanding of these mechanisms, made possible by a combination of first-rate experimental and numerical results, will enable us to optimize these new particle and light sources.
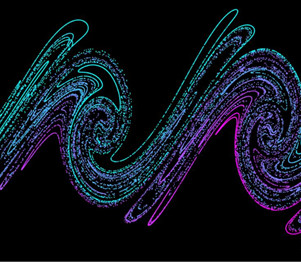
Read more
Time-compression methods for high-power laser pulses enable us to obtain femtosecond pulses (10-15 s) and thus reach extreme intensities (I >1018 W.cm-2), with which we can explore light-matter interaction under hitherto unknown conditions. When such laser pulses are focused on a solid target, the latter is almost instantaneously ionized at the surface, forming an electron-dense plasma that reflects the incident laser field.
In the present study, a collaboration between researchers at CEA Saclay and LBNL reveals that the crucial parameter for describing the coupling mechanism between a laser pulse and a plasma in the relativistic regime is the gradient length L, characteristic of the spatial expansion of the plasma. The value of L can be adjusted by taking part of the main pulse to obtain a pre-pulse of well-defined intensity, which following a shorter optical path, can reach the target before the main pulse and with an adjustable advance. This first pulse creates an initial plasma, the density and expansion of which can be finely controlled. Better control of the quality of the two successive high-contrast laser pulses has enabled the researchers to highlight two quite distinct regimes of plasma coupling with the main laser pulse, one occurring for gradient lengths L much shorter than the laser wavelength (L WARP/PICSAR code subsequently elucidated the two plasma absorption mechanisms.
At short L gradient lengths, the laser field reflects off a still confined and very dense plasma, which acts as a “plasma mirror“. In this regime, electrons are periodically expelled by the laser field and then re-accelerated back into the plasma, absorbing a significant fraction of the laser intensity. This mechanism is known as the “Brunel mechanism“.
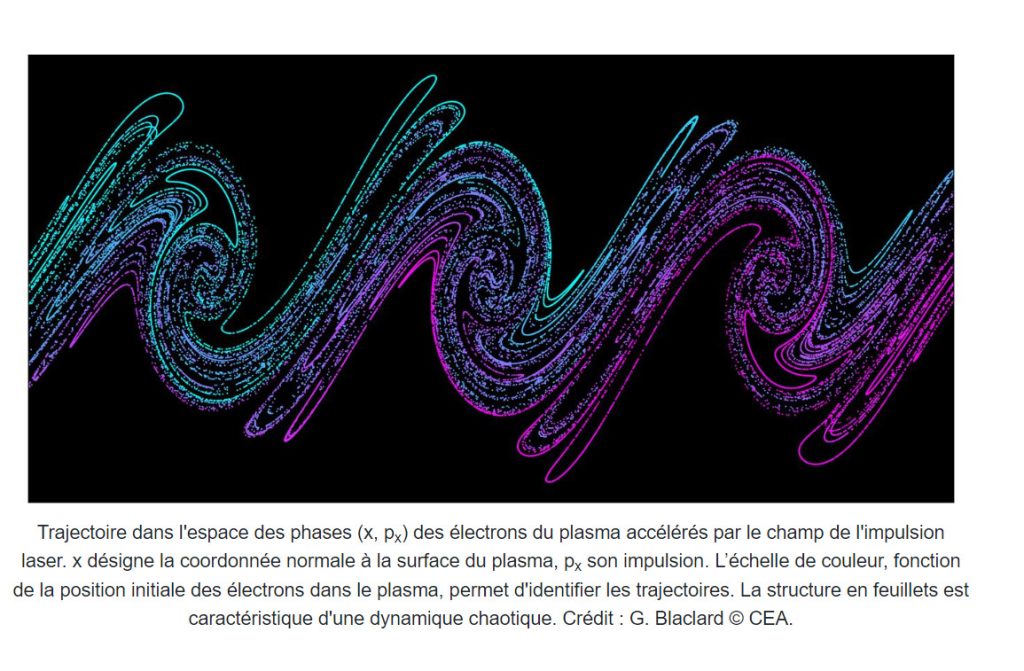
For higher values of L, of the order of the laser wavelength, a good proportion of the electrons at the vacuum-plasma interface are in a sub-critical density zone, where the incident field can propagate without reflection. They then find themselves subject to the interference field between the incident laser field and the laser field reflected from the denser, inner part of the plasma. In this regime, electron dynamics become chaotic and the electrons are heated by stochastic heating. This process is illustrated in the figure above, which shows the trajectories of plasma electrons in phase space (x, px) during interaction: x represents the coordinate normal to the plasma surface, and the color scale corresponds to the initial position of the electrons in the target, enabling their trajectories to be identified. This phase space shows a stretching and folding dynamic, also known as ‘optical kneading’, responsible for the appearance of sheet-like structures that are a clear signature of the emergence of chaos during interaction. As a result of these dynamics, two electrons initially close in phase space exhibit singularly different trajectories within a few optical cycles.
These results demonstrate the major role played by the gradient length L in the coupling mechanism. The detailed understanding of these mechanisms, made possible by the combination of experimental and numerical results, will enable us to optimize the particle and light sources resulting from laser-plasma interaction, with their multiple applications.
Publication
L. Chopineau, A. Leblanc, G. Blaclard, A. Denoeud, M. Thévenet, J-L. Vay, G. Bonnaud, Ph. Martin, H. Vincenti, and F. Quéré,
Phys. Rev. X 9 (2019) 011050.
Network targets
Resonant surface-wave excitation to boost harmonic generation on plasma mirror
As the UHI100 platform enables us to work with intense laser beams with high temporal contrast, we have been able to explore the dynamics of laser-matter interaction in the relativistic regime on structured targets(“lattice” plasma mirrors) and have observed for the first time experimentally an enhancement of surface plasmon (SP)-induced high-order harmonic generation on lattice targets subjected to relativistic intensities.
Read more
Our results reveal the significant role of SP excitation in reducing the intensity loss experienced by lattice-dispersed HHGs. This phenomenon not only increases the efficiency of the generation of certain harmonic orders, but also preserves their angular separation with respect to the laser’s fundamental frequency. The simultaneous acceleration of the electrons testifies to the persistence of plasmonic effects at relativistic intensities, even in the presence of the controlled density gradients required to achieve efficient HHG generation. This introduces a new dimension to our understanding of the relativistic resonant excitation of laser-generated surface plasma waves, promising new insights into the complex interaction between light and matter at extreme intensities.
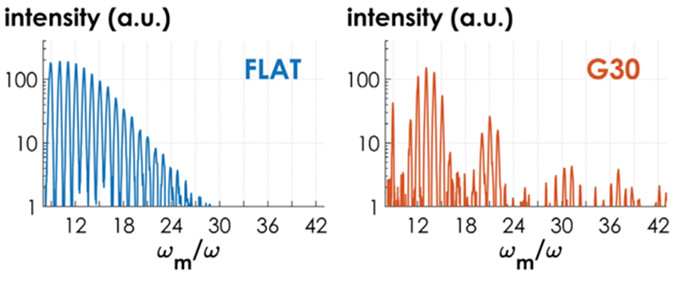
On the left, the harmonic spectrum obtained from a simple flat target (FLAT), collected in the direction specular to that of the incident laser (45°). On the right, the same laser is focused on a grating target (G30) at resonance angle (~35°) and collected in a direction close to the tangent to the target (87°). The spectrum from the G30 target is composed of higher-order harmonics than on the planar target (∼ 40). Some harmonics are as high in intensity (harmonics 12 to 15) or even higher (harmonics 18 to 21) than those obtained with the flat target. Intensity modulation is caused by diffraction, for which only harmonics that satisfy the grating equation are emitted along the chosen observation direction.
MP as a high-charge electron injector (VLA)
Plasma mirrors: source of relativistic high-charge electrons laser-accelerated in a vacuum
At ultra-high intensities (I>1016 W/cm2), the response of the plasma mirror (MP) to the laser field becomes highly non-linear, leading to the generation of high-order harmonics of the incident laser frequency in the reflected beam, associated in the time domain with a train of intense atttosecond pulses. The PHI group has been exploring this topic for some fifteen years now. The main mechanisms leading to this emission, Coherent Wake Emission (CWE) on the one hand, and the Relativistic Oscillating Mirror (ROM) on the other, are well identified and qualitatively understood.
Correlated with harmonic emission and close to the direction of the laser reflected in the specular direction, relativistic electron beams (≈ 10 MeV energy for I = 3 × 10¹⁹ W/cm², see Fig. 1) are also accelerated in the vacuum direction. To date, experimental studies of this electron emission under ultra-intense laser field (UHI) conditions remain limited, with the result that the underlying mechanisms remain poorly understood.
We have recently revealed a characteristic feature of this emission [The15].
Related publication
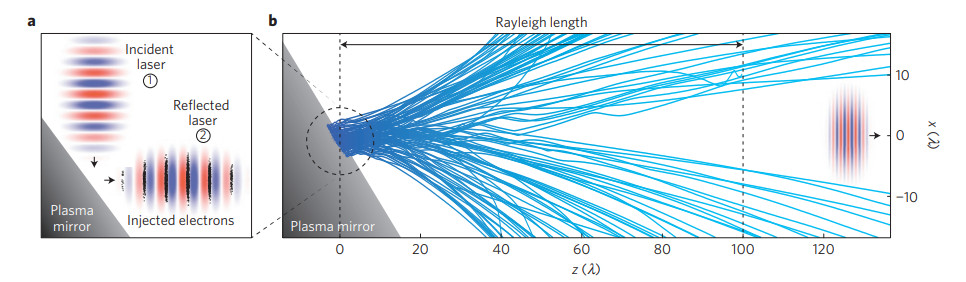
Injecting relativistic electrons into ultra-intense laser fields using plasma mirrors. Panel a): principle of a plasma mirror injector. When an ultra-intense laser beam (electric field outlined in red and blue) reflects off a plasma mirror, it expels relativistic electrons (black dots) at specific phases of the field. These electrons then interact with the reflected beam in vacuum. Panel b) shows the electron trajectories (blue lines) calculated from a 2D PIC simulation of the laser-plasma interaction. The electrons were initially located close to the surface of the plasma mirror. Once expelled from the surface, they co-propagate and interact with the reflected laser field over a distance of the order of the Rayleigh length. This interaction clearly alters the angular distribution of the electrons, which are expelled to the sides of the focal volume.
Read more
Electrons are ejected from the plasma surface in attosecond packets, with relativistic velocity following the direction of the laser field reflected in the specular direction. These electrons then co-propagate with the reflected laser beam in vacuum, interacting with it over distances of the order of the Rayleigh length. The spatial pattern observed on the electron beam (see figure 2) contains clear signatures of this interaction, and constitutes one of the most direct proofs to date of processes such as Vacuum Laser Acceleration (VLA), i.e. the direct acceleration of electrons by light in a vacuum, resulting in a luminous peak in the electron beam close to the specular direction) and electron scattering by the relativistic weight-motive force (which gives rise to a “hole” in the electron beam around the specular direction). By acting as electron injectors in the laser field, with charges measured in the 10 nC range, plasma mirrors thus open the way for the first time to studying the dynamics of free relativistic electrons in ultra-intense laser fields. This is a subject of great fundamental interest, having motivated considerable theoretical work in recent decades, but which until the mid-2010s remained experimentally inaccessible.
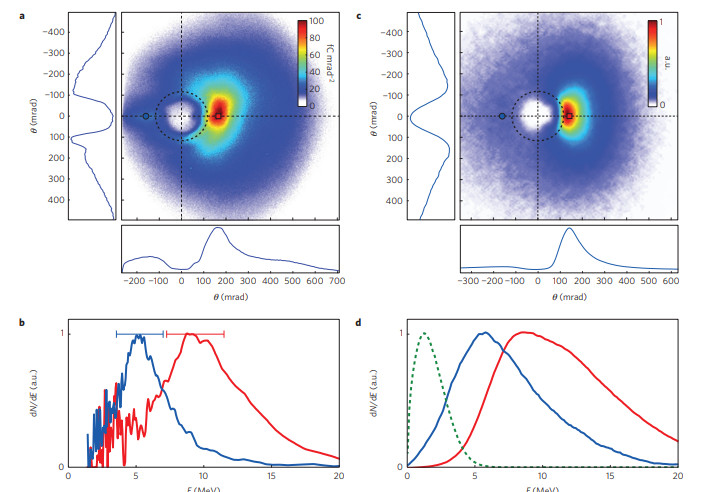
Experimental evidence for laser acceleration in vacuum. Figure a) shows a typical experimental angular distribution of electrons emitted by plasma mirrors in vacuum. This distribution consists of a broad emission cone (blue disk), strongly modulated by two main patterns. The first is a well-defined hole (in white) around the reflected laser beam, whose size and position in the detection plane are indicated by a dotted circle, resulting from the ejection of electrons from the laser axis by the weight-motive force after their ejection from the plasma mirror. The second pattern is an intense peak (in red), right at the edge of this hole, due to the laser acceleration in vacuum of a fraction of these electrons. Figure b) shows the electron spectra measured at two distinct locations in the beam. These locations are indicated by a blue circle and a black square in figure a), corresponding respectively to the blue and red curves in figure b). Figures c) and d) show the same data, but obtained from numerical simulations based on a 3D model of the test particles. The dotted curve in figure d) represents the initial electron energy distribution used in this model.
Effective acceleration of electrons in vacuum with a longitudinal laser field
Researchers from the LIDYL group and LOA(Laboratoire d’Optique Appliquée, ENSTA-X-CNRS) have succeeded for the first time in accelerating electrons by vacuum laser acceleration (VLA) to relativistic energies using a longitudinal electric field. To achieve this result, the linear polarization usually used in experiments was converted to radial polarization, which has an ideal structure for vacuum laser acceleration and produces better collimated electron beams.
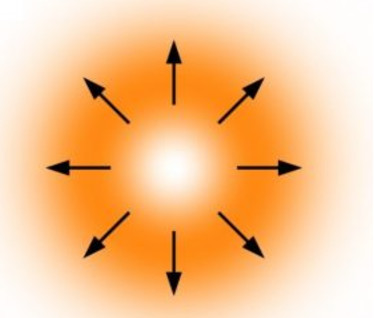
Space-time compression of harmonics
First measurements of spatio-temporal compression of harmonics generated on Miroir Plasma
In 2019, three-dimensional PIC (Particle In Cell) simulations predict that it is possible to increase the illumination of a laser after reflection on a plasma mirror (PM), which is capable of spatio-temporally compressing the initial pulse. Experimental validation of these predictions is a major challenge for the community. In 2021, the PHI team will be verifying this experimentally with measurements carried out on the UHI100 platform, enabling the spatio-temporal characterization of ultrashort harmonic pulses (HHG) emanating from MPs. To achieve this, an original, innovative method was developed during L. Chopineau’s thesis, based on ptychography (a lens-free imaging method), with the addition of a dynamic component. The difficulty and originality of the method lies in the creation of a diffracting object
that can be modulated temporally on the attosecond scale and spatially on the scale of the incident laser’s footprint on the MP, i.e. a few microns. A transient optical grating, which evolves continuously along the surface of the MP, is thus created thanks to a second beam of different frequency from the main beam, which interferes with the latter, is the diffracting object.
Experimental measurements, coupled with numerical simulations, have enabled us to deduce that the HHGs are focused some 150µm beyond the curved surface of the MP and that the gain in intensity, on a 100TW class laser such as UHI100, is close to an order of magnitude, making it possible to reach here the order of 1020W/cm2.
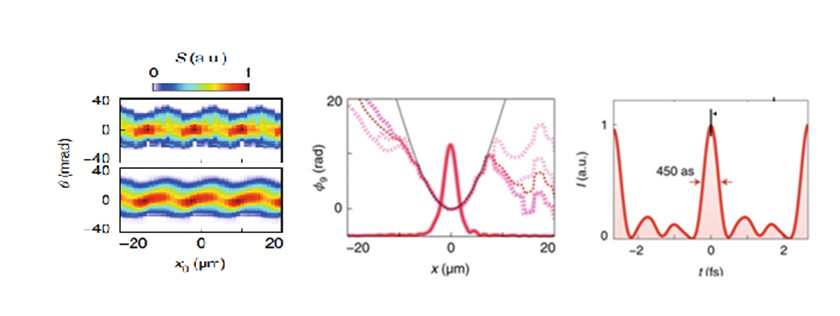
Left: frequency-resolved ptychographic traces obtained in the far field, sweeping dt=x0/v, where dt is the delay between the main beam and the auxiliary beam (above: experiment, below: reconstruction using the iterative “phase-retrieval” algorithm; center: Spatial phase and amplitude for Harmonic 9 (H9); right: spatially averaged temporal reconstruction of the field (Harmonics 9 to 14) showing a pulse width of 450as
Approaching the Schwinger limit with relativistic plasma mirrors
One of the major challenges in high-field physics is to develop a light source capable of reaching intensities >1025W.cm-2, at which high-field quantum electrodynamic (QED) effects become dominant in laser-matter interaction. In particular, at around 1029W.cm-2 (Schwinger Intensity), the laser field could literally ‘break the vacuum’ and produce e-/e pairs right in the vacuum.
However, these intensities are more than 3 orders of magnitude higher than the maximum intensity delivered by current PW lasers (1022W.cm-2). Reaching them is therefore impossible with current laser technology and therefore requires a paradigm shift that we have proposed
(i) focusing the reflected field on much smaller spot sizes than those possible with the incident (longer-wavelength) field.
This implementation uses relativistic plasma mirrors (PMs) optically curved by radiation pressure, which can be obtained by focusing a high-power femtosecond laser with very high contrast onto a solid target (Fig. a). At focus, the laser field causes the MP to oscillate at relativistic velocities and curves its surface by radiation pressure due to its inhomogeneous spatial profile (the field is stronger at the center of the focal spot than at its edges).
First-principles 3D Particle-In-Cell (PIC) simulations carried out using our WarpX code on the MIRA supercomputer (20 Mh CPU-800k cores) demonstrate that intensities of the order of 1025W.cm-2 (Fig.b-f) can be achieved at the focus of the plasma mirror for a 3PW incident laser (initial intensity 1022W.cm-2).
As these interaction conditions are experimentally accessible, this new implementation of the CRM concept should soon enable major advances in strong-field science over the coming years.
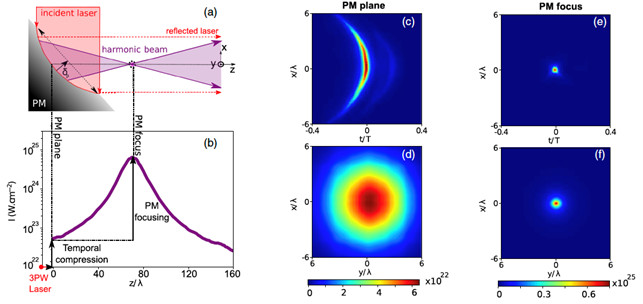
3D Particle-In-Cell (PIC) simulation of the focusing of the field reflected by a relativistic plasma mirror optically curved by radiation pressure (a) Schematic diagram of the interaction (b) Intensity of the reflected field as a function of distance from the plasma mirror (c) Spatio-temporal profile of intensity in theintensity profile in the plane of the plasma mirror (d) Spatial profile of the reflected field in the plane of the plasma mirror (e) Same as (c) but at the focus of the plasma mirror (f) Same as (d) but at the focus of the plasma mirror.