Introduction
We study the acceleration mechanisms of electrons and protons triggered by the interaction of a very high intensity laser pulse (>1018 W/cm2, also known as the relativistic regime) with solid or gaseous targets. The particle sources thus created have remarkable properties of brevity, charge, energy and emittance, opening up new perspectives in the fields of gas pedal physics, radiobiology and radiotherapy, as well as imaging.
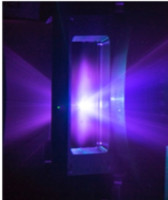
Structured targets
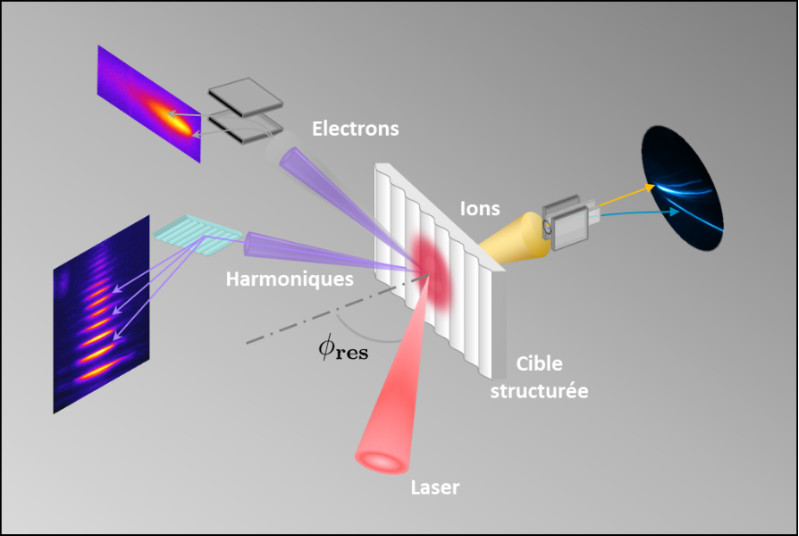
The interaction of a laser pulse in the relativistic regime, i.e. at intensities in excess of1018 W/cm2, with a solid or gaseous target generates energetic and brilliant secondary radiation sources, which have been studied for decades as possible alternatives to conventional gas pedals.
At such high intensities, all irradiated material is transformed into plasma within a few optical cycles of the laser pulse. The resulting free electrons can reach near-light speeds, with energies ranging from a few MeV to a few GeV, depending on the plasma density and the relative interaction regime.
Read more
In this context, “supercritical” plasmas are defined as those whose density exceeds the critical density, i.e. the density at which the plasma becomes opaque at the wavelength of the laser used. Typically, these plasmas, whose density is comparable to the density of a solid (ne ≥ 1023 cm3), are obtained in the laboratory by interacting a very intense, short-duration laser beam with a solid target. Long confined almost exclusively to the generation of proton sources
This is due to the exploration of new interaction regimes leading to the generation of more energetic, more numerous and, in some cases, more directional electrons.
One of the most popular strategies is to use micro-structured targets, in particular diffraction gratings. The coupling of this type of target to the laser’s electromagnetic field excites surface plasmons, which are collective electron oscillations at the interface between two materials with different dielectric constants.
In the relativistic interaction regime, surface plasmons are excited when the laser irradiates the grating surface at a certain angle of incidence, known as the resonance angle, which depends mainly on the grating pitch. From an experimental point of view, the temporal contrast of the laser must be sufficiently high so that the pedestal intensity, produced by the amplification of spontaneous emission along the laser chain, does not erase the periodicity of the target before interaction with the peak of the pulse. However, the recent development of techniques for enhancing laser contrast, such as the plasma mirror used on the UHI100 chain, has made it possible to use micro-structured targets in high-intensity laser-plasma interaction.
In this context, experiments carried out at the CEA (Saclay, France) have explored the resonant excitation of surface plasmons at relativistic intensities, paving the way for the whole new field of Relativistic Plasmonics
Surface plasmon-induced electron acceleration stands out for its robustness and ease of implementation.
Moreover, the non-linear interaction between the laser pulse and the supercritical plasma also results in the generation of harmonics of the laser frequency. In particular, plasma electrons oscillating across the vacuum interface reflect the incident laser field and cause a frequency shift by the Doppler effect.
This is what we have demonstrated with the very first experimental observation of this effect: the excitation of a surface plasmon turns out to be correlated with an increase in the harmonics emitted along the lattice tangent (up to ≥ 35!)
Laser-plasma gas pedal
Focused in a gas, the short, ultra-intense pulses (1018-1019W/cm2) available today instantly ionize the medium, transforming it into a plasma and generating relativistic electron beams with remarkable properties: short duration (fs), low divergence (mrad), high energy (several GeV). The electric fields generated during interaction reach hundreds of GeV/cm over centimetric acceleration distances. By way of comparison, conventional gas pedals can reach these energies, but over distances of several kilometers!
Since the first experimental demonstration of quasi-monoenergetic electron spectra in 2004 (see Nature 431), the field of laser plasma gas pedals (LWFA) has made enormous progress, benefiting from the constant evolution of laser technologies. While the physics of intense laser-gas coupling is increasingly well understood, the current challenges are to develop sources with optimized parameters for targeted applications, to move towards the development of machines, and to move towards ever-higher energies.
For the past ten years, we have been developing and testing targets on our UHI100 platform and on the APOLLON IR for laser-plasma acceleration, and more recently for radiotherapy applications.
Read more
ELISA target – variable-length gas cell for laser-plasma acceleration
ELISA is a gas cell developed in collaboration with LPGP for use on 100TW class lasers (UHI100, LLC) as well as on APOLLON (comissioning of the LFA room). The study of electron beam properties on various laser systems enables us to :
- generate electron beams and transport them via a magnetic system designed by IRFU as part of the EquipEx CILEX[A. Maitrallain, NIMA, 2018 ] . This paves the way for applications in radiotherapy
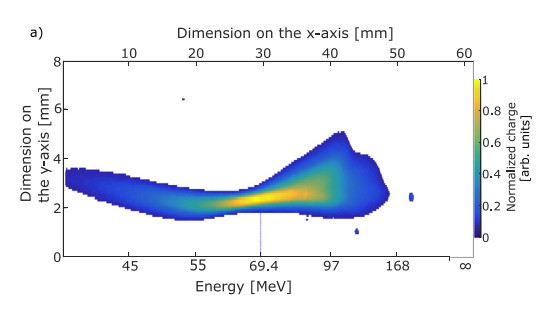
Electronic spectrum obtained on the LANEX screen placed in the focal plane of the magnetic transport line, 981mm after the laser focusing plane.
- participate in the start-up of the long-focus room on the APOLLON IR by generating electron beams of qq 100aines de MeV. This first experiment, carried out as part of the EquipeX CILEX, and piloted by B. Cros (LPGP), highlighted the influence of laser parameters such as the asymmetry of the transverse energy profile of the laser beam and laser point fluctuations in the focal plane, which degrade the charge and energy properties of the electron beam[I. Moulanier, PoP, 2023]
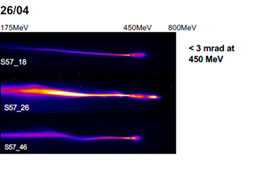
Examples of electron spectra obtained in LFA with a 6mm long cell
- characterize the emittance of a positron source generated on the UHI100 platform, by converting the primary electron beam generated by the ELISA cell into a high-Z target[A. Alejo, PPCF, 2020].
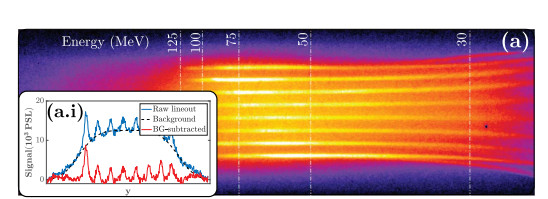
Raw image of the secondary electron beam as it passes through the mask and magnetic dipole (pepper pot technique), then analyzed for indirect characterization of positron beam emittance
The experiment was used as a test bench before being carried out on the Apollon IR* with G. Sarri’s team (Queen’s University, Belfast), LPGP, LLR and LULI. This made it possible to validate the operation of a gamma-ray detector, triggered by the Bremsstralhung emission generated by the interaction of the electron beam produced at 1.7GeV maximum, for around 207 /-62pC on a 1mm-thick Tantalum target. [N. Cavanagh, PRR, 2023]
Publications
“Gas cell density characterization for laser wakefield acceleration”
Audet TL, Lee P, Maynard G, Dobosz Dufrénoy S, Maitrallain A, Bougeard M, Monot P and Cros B, Nuclear Instruments and Methods in Physics Research Section A: Accelerators, Spectrometers, Detectors and Associated Equipment, January, (2018).
A.Maitrallain et al, NIMA, 2018 = Transport and analysis of electron beams from a laser wakefield accelerator in the 100 MeV energy range with a dedicated magnetic line“
Maitrallain A, Audet TL, Dobosz Dufrénoy S, Chancé A, Maynard G, Lee P, Mosnier A, Schwindling J, Delferrière O, Delerue N, Specka A, Monot P and Cros B, Nuclear Instruments and Methods in Physics Research Section A: Accelerators, Spectrometers, Detectors and Associated Equipment, November, 2018. Vol. 908, pp. 159-166, (2018).
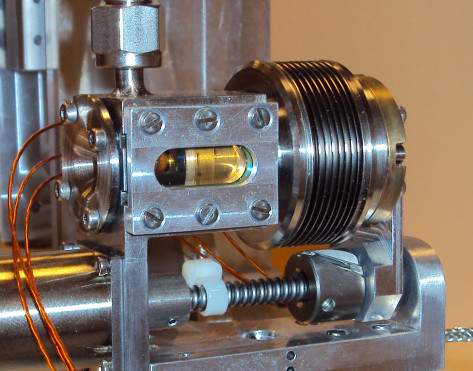
Plasma mirror as a high-charge electron injector (VLA)
Plasma Mirrors: source of relativistic high-charge electrons laser-accelerated in vacuum
At ultra-high intensities (I>1016 W/cm2), the response of the plasma mirror (PM) to the laser field becomes highly non-linear, leading to the generation of high-order harmonics of the incident laser frequency in the reflected beam, associated in the time domain with a train of intense atttosecond pulses. The PHI group has been exploring this topic for some fifteen years now. The main mechanisms leading to this emission, Coherent Wake Emission (CWE) on the one hand, and the Relativistic Oscillating Mirror (ROM) on the other, are well identified and qualitatively understood.
Correlated with harmonic emission and close to the direction of the laser reflected in the specular direction, relativistic electron beams (≈ 10 MeV energy for I = 3 × 10¹⁹ W/cm², see Fig. 1) are also accelerated in the vacuum direction. To date, experimental studies of this electron emission under ultra-intense laser field (UHI) conditions remain limited, with the result that the underlying mechanisms remain poorly understood.
We have recently revealed a characteristic feature of this emission [The15].
Related publication
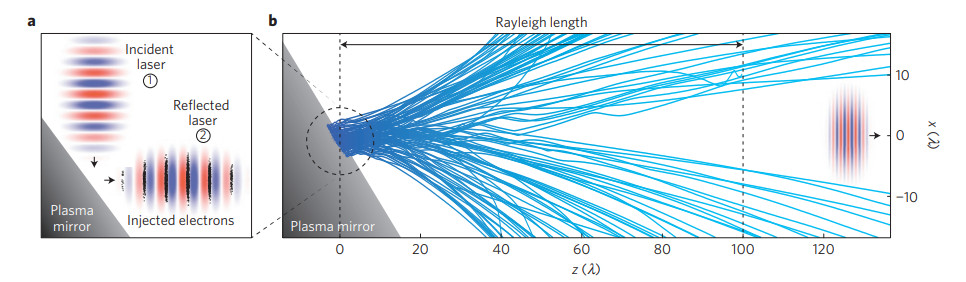
Figure 1: Injection of relativistic electrons into ultra-intense laser fields using plasma mirrors. Panel a): principle of a plasma mirror injector. When an ultra-intense laser beam (electric field outlined in red and blue) reflects off a plasma mirror, it expels relativistic electrons (black dots) at specific phases of the field. These electrons then interact with the reflected beam in vacuum. Panel b) shows the electron trajectories (blue lines) calculated from a 2D PIC simulation of the laser-plasma interaction. The electrons were initially located close to the surface of the plasma mirror. Once expelled from the surface, they co-propagate and interact with the reflected laser field over a distance of the order of the Rayleigh length. This interaction clearly alters the angular distribution of the electrons, which are expelled to the sides of the focal volume.
Read more
Electrons are ejected from the plasma surface in attosecond packets, with relativistic velocity following the direction of the laser field reflected in the specular direction. These electrons then co-propagate with the reflected laser beam in vacuum, interacting with it over distances of the order of the Rayleigh length. The spatial pattern observed on the electron beam (see figure 2) contains clear signatures of this interaction, and constitutes one of the most direct proofs to date of processes such as Vacuum Laser Acceleration (VLA), i.e. the direct acceleration of electrons by light in a vacuum, resulting in a luminous peak in the electron beam close to the specular direction) and electron scattering by the relativistic weight-motive force (which gives rise to a “hole” in the electron beam around the specular direction). By acting as electron injectors in the laser field, with charges measured in the 10 nC range, plasma mirrors thus open the way for the first time to studying the dynamics of free relativistic electrons in ultra-intense laser fields. This is a subject of great fundamental interest, having motivated considerable theoretical work in recent decades, but which until the mid-2010s remained experimentally inaccessible.
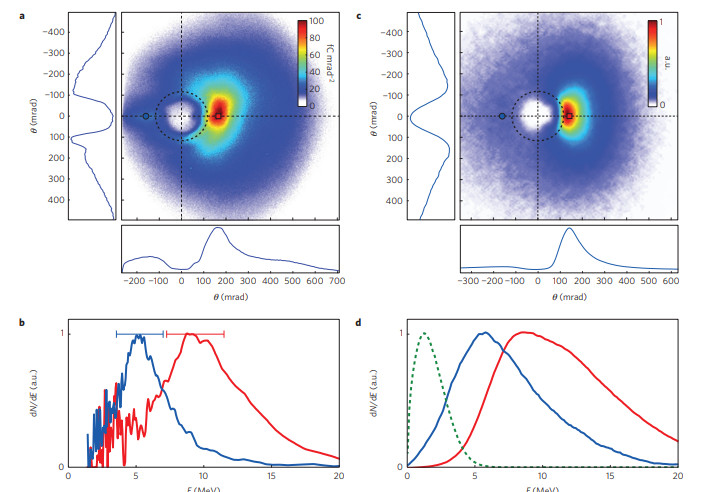
Figure 2: Experimental evidence for laser acceleration in vacuum. Figure a) shows a typical experimental angular distribution of electrons emitted by plasma mirrors in vacuum. This distribution consists of a broad emission cone (blue disk), strongly modulated by two main patterns. The first is a well-defined hole (in white) around the reflected laser beam, whose size and position in the detection plane are indicated by a dotted circle, resulting from the ejection of electrons from the laser axis by the weight-motive force after their ejection from the plasma mirror. The second pattern is an intense peak (in red), right at the edge of this hole, due to the laser acceleration in vacuum of a fraction of these electrons. Figure b) shows the electron spectra measured at two distinct locations in the beam. These locations are indicated by a blue circle and a black square in figure a), corresponding respectively to the blue and red curves in figure b). Figures c) and d) show the same data, but obtained from numerical simulations based on a 3D model of the test particles. The dotted curve in figure d) represents the initial electron energy distribution used in this model.
Effective acceleration of electrons in vacuum with a longitudinal laser field
Researchers from the LIDYL group and LOA (Laboratoire d’Optique Appliquée, ENSTA-X-CNRS) have succeeded for the first time in accelerating electrons by vacuum laser acceleration (VLA) to relativistic energies using a longitudinal electric field. To achieve this result, the linear polarization usually used in experiments was converted to radial polarization, which has an ideal structure for vacuum laser acceleration and produces better collimated electron beams.
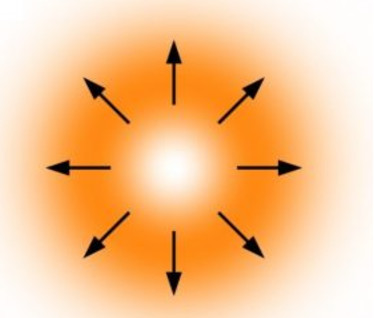
Hybrid target
New high-charge injector concept for plasma mirror-based laser-driven gas pedals
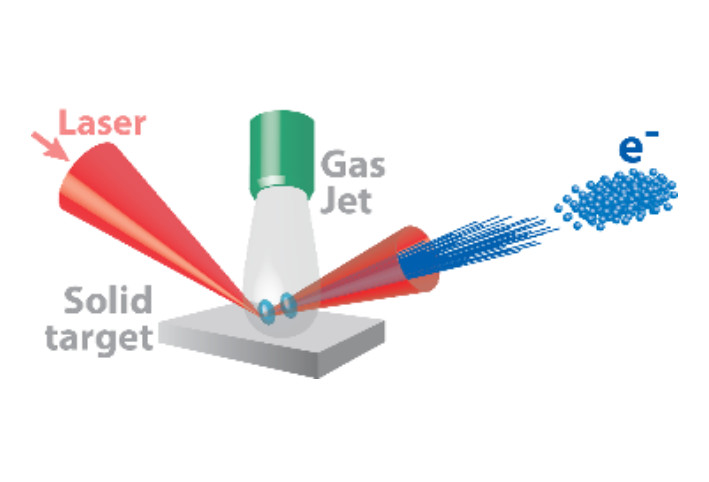
Laser-driven gas pedals (better known as laser-plasma gas pedals) are very promising candidates for providing ultra-compact, high-dose-rate gas pedals for applications in medicine, industry and high-energy physics. However, these gas pedals suffer from significant limitations, notably a lack of high-energy loading. To overcome this limitation, we have
developed, using massively parallel numerical simulations, a new injector concept that increases the charge delivered by laser-plasma gas pedals by almost an order of magnitude, with the aim of making these gas pedals suitable for use in applications. This concept is the subject of a patent filed jointly with CNRS under ref. FR2203449 (2022).
Read more
This new injection scheme involves focusing a high-power laser on a solid target coupled with a gas jet to accelerate more charge, while preserving beam quality. At laser focus, the solid target is fully ionized, forming a dense plasma that reflects the incident field: a “plasma mirror”.
As the laser reflects off this plasma mirror, a lot of charge can be injected from its surface into the “accelerator bubble” formed in the wake of the reflected field (see figure below).

Fig. – (a-b-c) – Snapshots from WarpX 3D simulations, before, during and after the reflection of an intense laser on a solid-gas hybrid target. The electron density of the gas is shown in orange-black scale, and the amplitude of the laser field in blue-red scale – (d) – Electron spectrum obtained after a 1mm propagation of the reflected laser in the gas part.
In 2022, we validated this concept
The resolution of the technical challenges that made these simulations possible, carried out in collaboration with our partners at LBNL, Riken, LOA, GENCI, ATOS and ARM, has enabled us to be awarded the Gordon Bell Prize for High-Performance Computing in 2022.
Publications
[1] L.Fedeli et al, SC22 Proceedings, 3, 1-12 (2022) https://dl.acm.org/doi/abs/10.5555/3571885.357188
Thomas Clark thesis (22/11/2024) – Université Paris Saclay – Plasma mirrors in laser-plasma gas pedals: towards high-charge, high-energy electron packets
Applications: FLASH radiotherapy
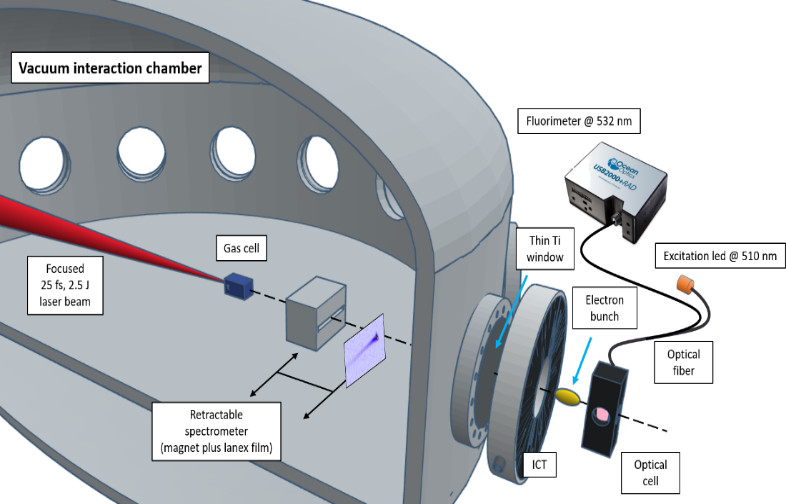
Radiotherapy (RT) is one of the most common tools used to treat many types of cancer. It uses ionizing radiation to destroy cancer cells and reduce tumor size. More than half of all cancer patients receive radiotherapy during their treatment (source WHO).
The major drawback of conventional RT is the intrinsic high-dose irradiation of healthy tissue surrounding the tumor to be treated, with the onset of undesirable short- and long-term side effects.
This is because RT uses an external radiation beam whose dose decreases exponentially as it passes through the patient’s body until it reaches the targeted tumor.
In recent years, FLASH RT, or the delivery of ultra-high radiation dose rates (UHDR) several orders of magnitude higher than those commonly used (~ 100 Gy/s vs. 5 Gy/min), has emerged as an irradiation modality that enables effective intervention on the tumor while reducing toxicity to surrounding healthy tissue.
Read more
Its use would thus increase dose and thus anti-tumor efficacy, with the added advantage of reducing treatment time. FLASH RT is currently recognized as one of the most promising breakthroughs in radiation oncology, at the crossroads of technology, physics, chemistry and biology.
By accelerating electrons through the interaction of intense, ultra-short laser pulses with plasmas (LPA), UHDRs can be produced with ease. These compact devices generate electron packets of very short duration (~fs) and very high energy (VHEE, 50-250 MeV). What’s more, unlike medical linear gas pedals, which operate at energies below 20 MeV, VHEEs offer a better dose/depth ratio than X-rays and, thanks to their penetration profile, can be used to irradiate most deep tumors in humans. Their use could also enhance the efficacy of FLASH therapy.
While the scientific community is in the process of tackling the problems inherent in LPA technology (reproducibility and precision of delivered dose, repetition rate, system reliability), the clinical transposition of FLASH radiotherapy nevertheless presents major challenges, particularly in terms of UHDR beam dosimetry.
In this regime (> 40 Gy/s), the detectors currently in use are subject to saturation effects, requiring the introduction of correction factors. Thanks to the experimental resources at our disposal, we decided to explore new methods of radiation detection and dosimetry. Our main objective is to develop a chemical dosimeter adapted to low pulse charge quantities and UHDRs (typically1012 Gy/s) for electron beams from LPAs. In particular, we propose a chemical dosimetry technique for VHEE based on the link between the intensity of fluorescence emission from an appropriately chosen molecule and the dose received.
“In the field of radiotherapy, the international community is buzzing with the beginnings of what could be a veritable revolution in the years to come”
Institut Gustave Roussy – Press release, November 2021